In the digital economy, trust has become the defining measure of credibility, influence, and commercial potential. Although online media platforms have removed geographical barriers and enabled unprecedented levels of connectivity, this very interconnectivity has also fostered new forms of deception, privacy violations, and reputational risks. In an age of high-profile data breaches, deepfake technology, and rapidly spreading misinformation, trust itself has emerged as the most valuable commodity. As users grow more wary, businesses, influencers, and content creators must reckon with how they establish, nurture, and safeguard the trust that underpins their ability to inspire loyalty and engagement.
Keyphrases: Digital Trust, Trust Building, Online Reputation Management, Consumer Perception in Digital Media
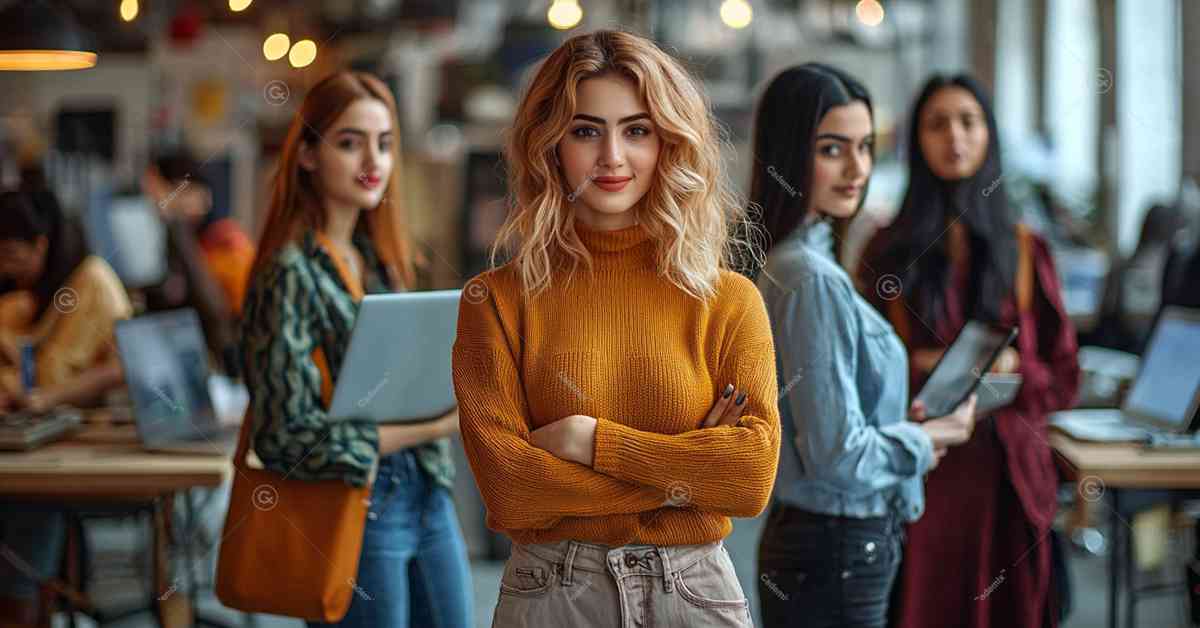
Abstract
Trust is often referred to as the glue of society, and nowhere is this more evident than in the digital realm. Because the internet enables the swift circulation of both accurate and misleading information, trust has become simultaneously a strategic advantage and a perpetual vulnerability. Organizations and influencers that succeed in building strong trust relationships can convert audiences into loyal brand advocates, while those who fail to address skepticism, data protection, or ethical concerns may see their credibility disintegrate overnight. This article examines the evolving concept of digital trust, the psychological and cultural factors shaping online perceptions, and the strategies through which brands can preserve authenticity in a world that often blurs fact and fiction.
Introduction
Trust has long served as a cornerstone of human relationships, whether in personal interactions or economic transactions. The difference in the digital age is the sheer velocity at which information is created, disseminated, and consumed. In physical contexts, trust typically forms incrementally through repeated interactions and consistent behavior—someone’s handshake, tone of voice, or day-to-day reliability can reinforce perceptions of integrity. Online, these subtle cues are either absent or easily fabricated, condensing trust formation into a matter of seconds.
Because so many aspects of modern life have shifted online—from socializing and shopping to professional networking and political discourse—trust has become an inescapable factor. The COVID-19 pandemic accelerated this transition, propelling much of the global population into remote workplaces, digital classrooms, and virtual social gatherings. Individuals found themselves relying on remote interactions not merely as conveniences but as indispensable tools for communication, commerce, and community support. As the internet has expanded into every facet of life, the cost of a trust breach—such as a hacked Zoom call or a compromised personal profile—has soared.
Further complicating matters is the challenge posed by anonymity and the absence of the shared reference points that shape trust in offline settings. The digital domain thrives on visual illusions and textual claims; without meeting face to face, users must rapidly determine the legitimacy of websites, social media profiles, or online marketplaces. This environment fosters skepticism and can trigger protective behaviors, with individuals frequently limiting the personal data they share or hesitating to engage wholeheartedly with unfamiliar platforms.
At the same time, business models anchored in advertising and data collection have thrust trust considerations into the spotlight. Consumers have grown more vigilant about how their data is harvested, used, or sold. Regulatory frameworks such as the European Union’s General Data Protection Regulation (GDPR) and the California Consumer Privacy Act (CCPA) were enacted to fortify user protections, but these regulations also underscore how fragile trust can be when it hinges on compliance and corporate transparency. In many cases, a single misstep—like an undisclosed data-sharing deal or the failure to protect user credentials—can send a brand’s reputation into freefall.
Misinformation compounds the complexity further. Social media algorithms prioritize content likely to generate high engagement, allowing sensational or dubious claims to circulate widely, sometimes overshadowing verified information. Deepfake technology has extended the potential for deception, enabling the production of realistic yet fabricated videos or images of public figures. The result is an atmosphere where users often question the authenticity of what they see, hear, or read online. For many brands, this climate poses a dual threat: not only must they contend with misinformation about their products or identity, they also must ensure their own content is perceived as genuine.
Yet this environment also yields opportunity. Brands that act responsibly, demonstrate transparency, and consistently meet user expectations can cultivate an enduring sense of reliability. Trust, in other words, becomes a competitive differentiator. Whether a brand is a small startup or an established multinational, the ability to create and maintain digital trust can influence consumer decisions, shareholder confidence, and partnership viability. As the subsequent sections illustrate, trust extends beyond surface-level marketing claims. It requires an ongoing commitment to authenticity, ethics, security, and responsive communication.
The Psychology of Digital Trust
Understanding how trust is formed in the digital sphere requires delving into the cognitive biases, emotional processes, and social triggers that govern human perception. The internet revolution may have changed the mediums of communication, but the fundamental mechanisms by which people decide whether to trust remain rooted in universal psychological tendencies.
One pivotal aspect is the reliance on heuristics: mental shortcuts that enable quick decisions about credibility. In an environment saturated with constant updates, alerts, and promotional messages, users do not have the bandwidth to scrutinize every claim. Instead, they gravitate toward signs that indicate reliability. Repetition can breed familiarity, leading individuals to trust brands they see consistently in their feeds or recommended by their peers. This is akin to the “mere exposure effect,” a phenomenon widely documented in cognitive psychology (see https://www.verywellmind.com/what-is-the-mere-exposure-effect-2795340 for additional discussion). When something appears often enough, it can appear more credible simply by virtue of repeated visibility.
Another key cognitive factor is the halo effect, in which one positive attribute (such as an association with a reputable individual or platform) radiates onto other aspects of perception. For instance, if a brand collaborates with a well-known influencer who is widely admired, the brand itself may inherit a portion of that credibility. Similarly, verified badges or endorsements from recognized institutions can instantly shift user perception from skepticism to acceptance. However, the opposite is also true. A negative association, such as a partnership with a questionable sponsor or a single high-profile scandal, can tarnish trust swiftly.
Social proof, a powerful driver of online behavior, also plays a significant role. People are more inclined to trust information that appears popular or widely endorsed, reflecting the concept that high engagement might indicate reliability. Customer reviews, testimonials, or even casual comments that praise a product can be persuasive, sometimes carrying more weight than professional critiques. Conversely, poor ratings or critical feedback on consumer review sites may deter prospective customers, regardless of official brand statements.
Emotions, too, govern how trust is either solidified or eroded. Positive emotions like optimism, excitement, or empathy can predispose a person to trust. Companies that inspire such emotions in their marketing campaigns often see amplified results. On the other hand, anger or fear can provoke suspicion, prompting individuals to doubt or question information. Brands that resort to manipulative fear tactics risk alienating large segments of their audience, raising concerns about their intentions or ethics. In practice, therefore, emotional resonance must be handled with care. Harnessing positivity can build trust, but stoking negative emotions may have unintended consequences.
In addition to these cognitive and emotional dimensions, digital trust hinges on perceived authenticity. Online, a brand’s or influencer’s “authentic self” often derives from how consistently they convey their mission, values, and style across multiple platforms. If there is a visible mismatch—a humorous, approachable tone on social media but a stiff, jargon-laden tone on the brand’s website—users may become uncertain which representation is genuine. This extends to the realm of personal branding, where individuals who share glimpses of their personal lives or vulnerabilities can seem more trustworthy than those who present overly curated personas.
Ultimately, the psychology of digital trust is about alignment. When a user’s expectations, prior experiences, and social environment converge in a way that validates a brand’s image or message, trust builds. When there are dissonances—mismatched claims, inconsistent content, or manipulative emotional appeals—trust can deteriorate. Maintaining this alignment necessitates not just marketing expertise but also a deep understanding of user psychology and a commitment to preserving integrity across every touchpoint.
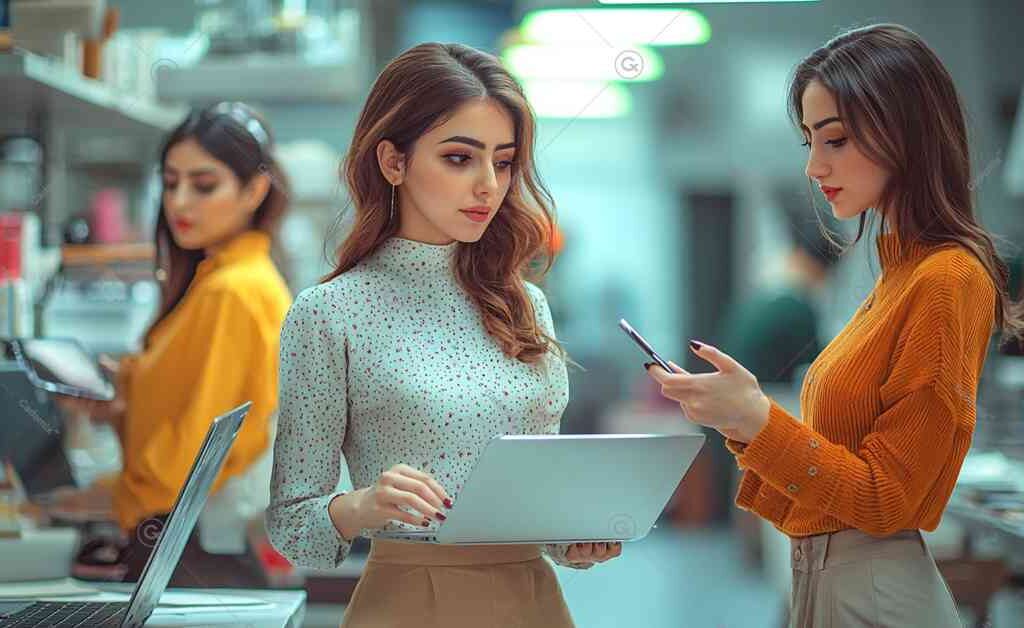
The Impact of Misinformation and Deepfake Technology
One of the defining traits of the digital era is the democratization of content creation. Anyone with an internet connection can publish, share, and amplify ideas to a worldwide audience. While this empowerment has fostered creativity and community-building, it has also facilitated the unchecked dissemination of false or misleading information. A single viral rumor on platforms like Twitter, TikTok, or Facebook can shape public discourse within hours, sometimes outpacing attempts at fact-checking or correction. This phenomenon is often called the “misinformation cascade,” a process wherein sensational claims gain traction faster than evidence-based refutations.
Such misinformation erodes digital trust, as it casts doubt on the reliability of almost any statement encountered online. Audiences accustomed to sensational headlines or conspiracies may grow cynical, questioning the legitimacy of genuinely credible sources. This environment poses a particular challenge for brands and influencers striving for authenticity. Even if a product is safe and well-verified, false rumors about side effects or unethical production methods can spread widely, leaving the brand to engage in a reactive, time-consuming effort to set the record straight. The reputational damage may linger, especially if users only recall the initial, dramatic claim rather than the subsequent retraction.
Deepfake technology raises the stakes further. Advanced AI techniques can generate lifelike videos or images depicting people doing or saying things they never actually did. Political figures, celebrities, and even private citizens can be inserted into scenarios they were never part of. Although various detection methods are evolving, deepfakes have already proven difficult to identify once they are circulated on social media. In high-profile cases, manipulated videos have sown confusion about real events, influencing public opinion and fueling conspiracy theories. The potential to undermine trust in legitimate footage is staggering: if people cannot be sure whether any given video is genuine, the very concept of “seeing is believing” collapses.
For businesses, deepfakes introduce new vulnerabilities. A competitor or disgruntled actor might fabricate damaging footage to tarnish a company’s reputation. Conversely, an organization’s marketing campaign might incorporate AI-generated content that straddles ethical lines, prompting backlash for perceived dishonesty. Content creators thus face a dilemma: how to harness emerging technologies without contributing to the erosion of trust.
Efforts to combat misinformation and deepfakes often pivot around technological, regulatory, and educational solutions. On the technological front, social media giants have rolled out detection algorithms designed to flag or remove suspect content, though these algorithms are not foolproof and can inadvertently take down legitimate material. Regulatory measures, such as mandates for labeling manipulated media, have been proposed in various jurisdictions, though enforcement remains sporadic. Some platforms have begun forming partnerships with independent fact-checking organizations to slow the spread of demonstrably false information. However, each approach has limitations and can spark controversy over free speech and censorship.
Media literacy and user education offer perhaps the most sustainable route to combating misinformation. By teaching people how to verify sources, check for consistent context, and remain skeptical of suspiciously viral claims, educational initiatives can empower audiences to serve as their own gatekeepers. Numerous NGOs and educational bodies provide open-source curricula on digital literacy, including the United Nations Educational, Scientific and Cultural Organization (UNESCO) and the International Federation of Library Associations (IFLA). When businesses and influencers also emphasize media literacy—linking to reputable fact-checks, clarifying data sources, and disclaiming potential biases—they model constructive practices that reinforce trust.
In essence, misinformation and deepfake technology highlight a broader truth about digital trust: it is never static and must be constantly guarded. In a climate where anything can be faked and everything can be doubted, the role of consistent truthfulness, transparency, and accountability stands out more than ever. Brands that shirk these responsibilities might temporarily gain attention, but they compromise their long-term credibility. Conversely, those that invest in robust verification and user education can emerge as bastions of reliability in an uncertain online world.
Strategies for Building and Maintaining Digital Trust
Because digital trust is simultaneously fragile and essential, brands and influencers cannot afford to approach it as a mere afterthought. A comprehensive strategy that addresses everything from user data protections to content authenticity can significantly bolster credibility. While there is no universal recipe, certain best practices have proven effective across a variety of industries.
One foundational element is transparency in data handling. Users are increasingly cognizant of how personal information might be exploited for targeted ads or sold to third parties. Clear privacy policies, explicit consent mechanisms, and minimal data collection can signal respect for user autonomy. For instance, e-commerce platforms that clarify how credit card information is stored and processed may see fewer abandoned carts and more repeat business. Similarly, content creators who disclose sponsorships and affiliate links upfront foster an environment of honesty, which can cultivate deeper trust over time.
Another pillar is consistent, humanized communication. People tend to trust brands that exhibit personality, responsiveness, and empathy. Social media profiles that function as two-way communication channels—responding to praise, addressing user concerns, and acknowledging errors—help audiences perceive the brand as accessible and genuine. This is especially critical during moments of crisis or controversy, when the silence or deflection can do far more damage than the original incident. By promptly accepting accountability for a mistake and detailing the steps taken to correct it, organizations can maintain goodwill even in challenging circumstances.
Demonstrable expertise reinforces trust. Whether it is a skincare brand publishing peer-reviewed studies on its product’s effectiveness or a tech influencer providing thorough tutorials and references, proof of knowledge and adherence to professional standards elevates a creator’s or company’s stature. Verified credentials, academic collaborations, or industry awards can also reassure audiences of authenticity. That said, attempts to feign expertise or inflate achievements almost invariably backfire once discrepancies become evident, resulting in sharper trust erosion than if the claims had never been made.
Security and reliability form another crucial layer. Data breaches not only harm customers but also severely damage an organization’s standing. Implementing robust cybersecurity measures, conducting periodic security audits, and adhering to certifications such as ISO 27001 for information security management can reassure stakeholders that the brand takes data protection seriously. Transparent reporting of potential vulnerabilities and timely notification about incidents can further underscore a brand’s commitment to user safety.
Fact-checking and content validation help address the misinformation challenge. When citing studies, referencing historical events, or making claims about a product’s benefits, it is prudent to link to credible sources. Using third-party auditing, such as professional fact-checking services or recognized certification bodies, can strengthen the reliability of published information. Even disclaimers that certain content represents opinion rather than fact can reduce confusion and ensure that audiences do not feel misled. By positioning honesty above short-term gains, an organization differentiates itself from competitors that may resort to half-truths or manipulative tactics.
Equally important is community engagement. Trust thrives in reciprocal relationships where audiences feel valued. Encouraging user-generated content, highlighting success stories from real customers, or hosting Q&A sessions can create a sense of co-ownership. This approach leverages social proof: when users see others interacting positively with a brand, they are more likely to do so themselves. Over time, an engaged community transforms into a microcosm of brand ambassadors, amplifying the message that this is a trustworthy entity worth supporting.
While implementing these strategies, consistent alignment between words and actions remains paramount. Declaring ethical intentions or commitment to quality is hollow if user experiences routinely contradict these declarations. In the same vein, maintaining trust requires perpetual upkeep and vigilance. The digital environment evolves rapidly; what satisfied transparency requirements a year ago may now be deemed insufficient given new regulations or heightened user awareness. Consequently, building a robust trust strategy is less about ticking boxes than about creating an adaptable, ethically grounded culture that stands the test of time.
The Future of Digital Trust
Looking ahead, the landscape of digital trust is poised to undergo further shifts in response to emerging technologies and changing cultural norms. Blockchain-based solutions, for instance, promise decentralized ways to verify identities, transactions, and even content authenticity. By relying on distributed ledgers, users may gain greater confidence that information has not been altered. These systems can also enhance supply chain transparency, allowing consumers to trace a product’s origin or track philanthropic donations without relying solely on corporate statements. However, such innovations also introduce their own complexities, such as blockchain’s energy consumption concerns and the need for user-friendly interfaces.
Decentralized platforms are beginning to challenge established social media models, offering communities more control over moderation and content ownership. This could redefine how trust is mediated, shifting power from corporate platforms to user-driven governance structures. While these experiments may help mitigate some issues related to algorithmic echo chambers, they also risk fragmenting online communities, creating scattered pockets of conversation that can be difficult to navigate. In such an environment, the ability to establish trust may depend on bridging multiple, sometimes insular networks.
Artificial intelligence will undoubtedly play a greater role in trust mechanisms. Machine learning models are already used for fraud detection, consumer recommendation systems, and spam filtering. In the future, AI-driven tools might analyze text or video content for inconsistencies, helping to flag potential deepfakes or manipulative posts. However, the transparency and fairness of these algorithms must be assured. If AI systems incorrectly label genuine content as deceitful, or if they disproportionately penalize certain voices, they can undermine trust in both the brand deploying them and the broader idea of AI-driven oversight. Building trust in AI systems thus becomes as critical as building trust in the brand itself.
Regulation will continue to evolve, as governments struggle to keep pace with the fast-changing digital realm. Laws addressing data protection, consumer privacy, and platform accountability may expand, forcing businesses to adapt or face penalties. On a global scale, differences in regulatory approaches can complicate compliance, requiring companies to tailor trust-building measures to diverse legal environments. This dynamic underscores the importance of flexible, principle-based ethics that transcend local rules and remain consistent across jurisdictions.
Cultural shifts also influence expectations around trust. Younger generations who grew up amid social media controversies and data scandals may demand more rigorous privacy controls and honest branding. Social movements around sustainability, corporate responsibility, and diversity inclusion shape the moral lens through which users assess a brand’s trustworthiness. A company that fails to address these broader societal expectations may come across as tone-deaf or outdated, especially if it tries to pass off superficial gestures as genuine commitments.
In the future, the concept of digital trust may be integrated into user interfaces through trust scores or badges, indicating how well an entity adheres to best practices. While such reputational markers could aid decision-making, they also risk oversimplifying complex ethical questions. Ultimately, the brands that navigate these shifts successfully will be those that embrace adaptability without sacrificing core values, that leverage new technologies for transparency rather than gimmicks, and that engage audiences in dialogue rather than monologue.
Conclusion
Digital trust stands as both a formidable barrier and a gateway to influence in an online environment saturated with information. It is formed quickly, can be lost in an instant, and must be re-earned continually through consistent, open, and credible practices. In a space where misinformation cascades feed cynicism and advanced manipulations like deepfakes cast doubt on even visual evidence, trust operates as the deciding factor that separates fleeting presence from enduring impact.
At a time when the currency of trust underwrites reputations, drives revenue, and galvanizes communities, brands and influencers who maintain transparent data policies, demonstrate real empathy in user engagements, and fortify their credibility with verifiable expertise establish themselves as beacons of reliability. They stand out precisely because trust has become so precarious and elusive. Their steadfastness can transform casual observers into brand advocates, cultivate strong consumer loyalty, and buffer them against potential crises.
The journey toward digital trust is never truly complete. It necessitates ongoing vigilance against evolving threats, from misinformation to data breaches, while also embracing emerging technologies that might strengthen authenticity. Above all, it calls for an ethical compass capable of guiding decisions in unsettled terrain. In this sense, trust is not just a marketing angle or a feature to be toggled on. It is the foundation upon which resilient digital relationships are built—a foundation that will only grow more critical as online interaction further intertwines with every aspect of modern life.
For additional resources on digital trust, consider exploring research from the Stanford Internet Observatory on disinformation, referencing guidelines on ethical AI from the Harvard Business Review, or reviewing privacy best practices outlined by the Electronic Frontier Foundation. By staying informed about these evolving conversations and tailoring strategies accordingly, businesses, influencers, and content creators can safeguard their most valuable intangible asset: the trust of the audiences they aim to serve.